HEART FAILURE (HF)
Motivation: Impairment of normal heart function (heart failure) afflicts 2% (~ 5-6 million) of the US population, and over 1 million new cases are reported annually, with healthcare costs exceeding $30 billion/year. Lack of donor hearts and increasing occurrences of heart failure have led to left ventricular assist devices (LVADs) transitioning from their originally intended purpose of bride-to-transplant to destination therapy (long-term heart failure treatment) devices. However, complications such as a high risk of stroke and formation of blood clots (emboli) continue to remain the major causes of mortality and morbidity in LVAD patients.
My research project aims at determining efficient, patient-specific LVAD implantation and management strategies for reducing stroke risk that will pave the way for long-term use of LVADs as destination therapy devices. Through this project, I aim to determine efficient, patient-specific cardiac device implantation strategies in collaboration with cardiothoracic surgeons. To achieve my goal, I plan to use state-of-the-art computational and experimental tools. I will perform virtual surgery and computational blood flow simulations to determine device implantation configurations that present minimal stroke risk in the long-term. Additionally, I will use the latest in 3D printing, rapid prototyping and experimental fluid mechanics techniques to create patient-specific flow phantoms to conduct bench-top experiments of computationally obtained implantation configurations.
Video showing flow patterns of blood exiting the LVAD outflow graft (left) into the ascending aorta, aortic arh, great vessels and descending aorta. Using CFD helps me in trying to determe an implantation configuration that poses the least risk of stroke.
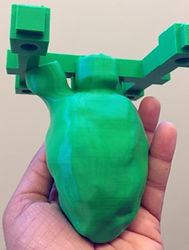
3D-printed patient-specific model of a left ventricle that is used to create flow phantoms for conducting bench-top experiments to investigate blood flow.
CEREBRAL ANEURYSMS
Motivation: Cerebral aneurysms can cause devastating brain hemorrhage, and treatment aimed at preventing rupture and hemorrhage is imperfect. There are approximately 30,000 aneurysmal hemorrhages in the United States each year, accounting for ~5% of all strokes. Over half result in death or disability; thus most unruptured aneurysms are treated to prevent rupture. The endovascular approach to aneurysm treatment, which employs minimally-invasive methods of treating aneurysms from within the brain blood vessels during cerebral angiography, is becoming the preferred approach to cerebral aneurysms. However, up to 34% of aneurysms treated this way are not cured. The overall goal of this project is to develop more accurate modeling techniques to inform clinical decision-making of aneurysm treatment to improve patient outcomes.
​
Some of my recent postdoctoral research focused on identifying hemodynamic factors that influence, and can hence predict, success or failure of cerebral aneurysm treatment in an attempt to improve treatment outcome and prevent brain hemorrhage. I am part of a team that includes engineers and neurosurgeons that measure intra-aneurysmal and surrounding vasculature hemodynamics in patients with intracranial aneurysms and using these measurements in high-fidelity patient-specific CFD investigations of hemodynamics pre- and post-treatment, we can elucidate the influence of treatment on specific hemodynamic factors which are predictive of treatment success or failure. Additionally, we reconstruct anatomically accurate, patient-specific flow phantoms of aneurysms and surrounding vasculature for performing Particle Image Velocitmetry (PIV) studies of flow patterns before and after treating the flow phantoms with the same treatment devices (coils and/or stents) as the actual patient.
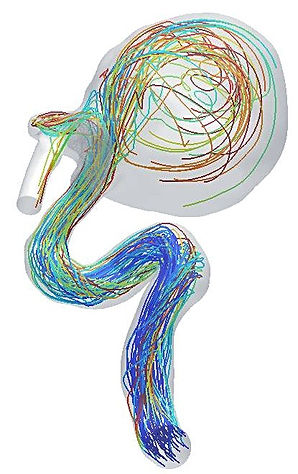
Representative trajectories of platelets traversing the cerebral vasculature and recieculating within the cerebral aneurysm sac in a patient

Anatomically accurate, true-scale patient-specific flow phantom created using 3D printing and rapid protyping. The model is "treated" by a neurosurgeon to obtain the morphology of the treating coil (shown inset) using high resolution scanning
ABDOMINAL AORTIC ANEURYSMS (AAA)
Motivation: The abdominal aorta is the part of the aorta (tube carrying blood) between the renal arteries (that provide blood to the kidneys) and the iliac arteries (that provide blood the lower body). Under some circumstances, the aorta can "bulge" and the integrity of the wall of the abdominal aorta is compromised. This "bulge" is called an aneurysm. As the aorta bulges, blood that flows through it can slow down and blood clots get deposited in the expanded portion, called a thrombus. If unchecked, this bulge can keep growing until a point where it may burst - this can be fatal. According to the CDC, AAA were responsible for around 17,000 deaths in 2009 in USA alone. My focus is to investigate the hemodynamic environment in the AAA including the stresses in the expanded wall and thrombus.
My research deals with patient-specific modeling of the biomechanics of AAA. USing CT-scans, I delineate the AAA, the thrombus and the lumen (the region where blood flows) and use techniques similar to those used in my research of congenital heart diseases (above) to generate patient-specific models for computational analysis. Using a combination of CFD to examine hemodynamics and computational solid mechanics (CSM) to examine the tissue environment, I can characterize the biomechanical state of the AAA. Working with medical doctors, I can help provide valuable insights in determining the best course of action for a patient with an AAA.
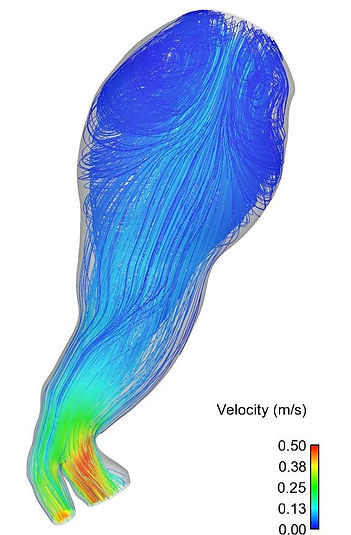
Blood flow through a large abdominal aortic aneyrusm (AAA). The lines depict the path taken by blood particles arriving in from the inlet (top). Recirculation zones (swirls) are clearly visible. Recirculating flow accelerates thrombus formation, thereby worsening the integrity of the vessel wall.
CONGENITAL HEART DISEASES (CHD)
Motivation: Around 1% of babies born in USA have some form of congenital heart disease, and most require invasive surgery within the first year. Fascinatingly, the human heart develops from a tube during the early embryonic stages. Research has shown that altered blood flow in a developing heart can lead to congenital heart disease. My focus is to determine the blood flow (hemodynamic) environment in developing embryos to investigate the effect of blood flow on cardiac development.
My research deals with subject-specific modeling of embryonic blood flow in chicken embryos using computational fluid dynamics (CFD). Since the developmental process in chickens is remarkably similar to humans, we can gain valuable insights from studying chicken embryos. Using experimentally obtained images, I generate subject specific models of the embryonic outflow tract (OFT), which gives rise to many cardiac structures such as heart valves and interventricular septum (wall). Using these models, I simulate blood flow within the OFT to characterize hemodynamic parameters such as pressure, velocity and wall stress distributions. These parameters are then compared between normal and abnormal embryos, thereby providing insights into developmental hemodynamics and their effects under abnormal circumstances.

Obtain images of embryonic heart outflow tracts (OFT)
Generate subject-specific computational models using image segmentation
Perfom CFD simulations to determine
blood flow dynamics
Pipeline for investigating congenital heart diseases
MICRO SCALE BLOOD CELL MODELING
Motivation: The heart contains valves to help direct the flow of blood within the different chambers (atria and ventricles). These valves work ceaselessly, undergoing a cycle of opening and closing every 0.8 seconds - thats about 100,000 times a day!! In some situations, these valves develop problems because of which they do not open and/or close properly. This can lead to severe problems, as it compromises the functionality and efficacy of the heart. There are roughly 500,000 cases of heart disease that are registered annually in USA alone.To counter the problem of dieseased heart valves, doctors implant mechanical heart valves (MHV) in place of the original tissue valves. These MHVs are essentially "doors" that open and close. As a result, blood flow through MHVs is drastically different than normal valves which open and close smoothly, like a cloth. The abnormal blood flow in MHVs gives rise to blood clots in and around the MHV, called thrombosis. Thrombosis can increase to very severe levels, and hence patients implanted with MHVs have to be on life-long anti-coagulation therapy, which affects their daily lives. My research focused on developing new methodologies to simulate the motion of individual blood cells and platelets in order to help understand the abnormal blood flow in MHVs and help provide design input to reduce the non-phyisological blood flow.
Even though thrombosis (blood clotting) occurs at the macro-scale (i.e. we can see it with our naked eyes), it is a phenomenon that is kick-started at the micro-scale (thats 0.000001 m!), i.e. at the level of individual blood cells and platelets. In order to understand the causes for thrombosis due to abnormal blood flow, we need to understand the mechanics of blood at the micro-scale. The human body has 2.5 Billion blood cells, and simulating blood flow even in a finite volume under physiological conditions is still impossible due to lack of computational capabilities. My research was based on the fundamental ideology that if we can develop a method to simulate a single cell very efficiently, we can use the existing computational resources to simulate a large number of cells to learn how they behave during abnormal flow.
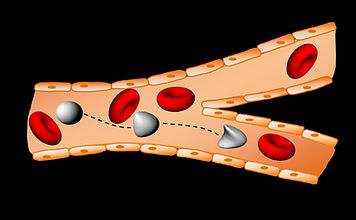
A schematic of multiple cells interacting with each other during flow in a capillary
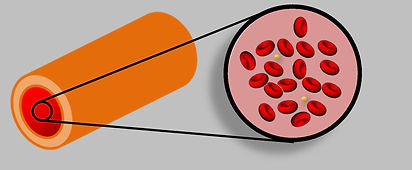
Zoomed-in view of blood, showing blood cells (red) and platelets (yellow). The artery (left) is at the macro scale (a level that we can see), while the blood cells are at the micro-scale (0.000001m).
Video showing the red blood cell model defroming in a typically observed flow in a capillary
CANCER METASTASIS
Motivation: Cancer is extremely dangerous primarily because of its ability to spread from a primary site to various other parts of the body. This spreading of cancer from primary to secondary sites is called "metastasis", which is responsible for over 95% of the deaths due to cancer. The most intriguing fact about cancer metastasis is that cancer cells use the blood circulatory network to spread to different parts of the body. With 2.5 Billion blood cells moving around, a lot of the cancer cells that enter the bloodstream actually die out due to collisions with blood cells and collisions with the walls of the arteries. It is only the cells that survive that actually end up spreading cancer. Even more intriguing is that cancer cells seem to sense the change in environment when they pass through the blood stream and somehow adapt to the very harsh hemodynamic environment in the blood vessels. My research focus was to try and determine if cancer cells did adapt to fluid flow, and if so, how?
Using simple experimental approaches, our research group at the University of Iowa passes a suspension of cancer cells through a very fine needle at physiological flow rates. This imparts fluid stresses on the cells, similar to what they may encounter in the blood stream. I performed mechanical testing on the cancer cells before and after passage through the needle to determine if they changed their mechanical properties after exposure to fluid shear. Sure enough, the cancer cells seemed to have "stiffened up" after exposure to fluid stresses, and this stiffening up appeared to be graded based on the flow rates. While a lot more research is required to determine the exact mechanisms by which cancer cells adapt to fluid stresses, my research provides valuable insights to hopefully, one day, devise methodologies to prevent cancer metastasising or render the metastasizing cells ineffective and unable to spread.
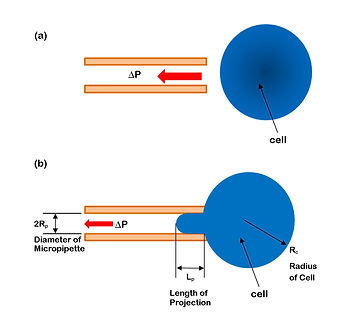
A schematic of how the micropipette aspiration experiment is performed. A small glass tube (micropipette) is manipulated towards a cell (a), and suction is applied to suck in part of the cell inside the micropipette (b). The extent of deformation of the cell depends on how much force it can withstand, thereby providing us with a measure of how strog a cell is.
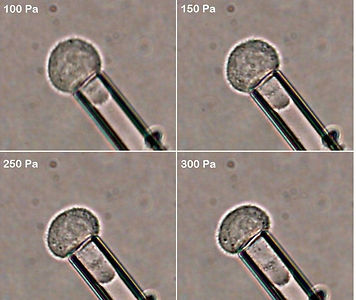
Snapshots of a typical experiment conducted by me on prostate cancer cells. The montage shows a prostate cancer cell being subjected to increasing stress - the deformation of the cell inside the micropipette can be clearly seen.
PUBLICATIONS
Publications:
​
-
V. Chivukula, JA Beckman, AR Prisco, TF Dardas, S Lin, JW Smith, NA Mokadam, A Aliseda and C Mahr, LVAD Inflow Cannula Angle and Thrombosis Risk, accepted for publication in Circulation: Heart Failure (2018)
-
TB Lawson, DE Scott-Dreschsel, VK Chivukula, S Rugonyi, K Thornburg, M Hinds, Hyperglycemia Alters the Structure and Hemodynamics of the Developing Embryonic Heart, Journal of Cardiovascular Development and Disease, 5(1), 13. (2018)
-
V. Chivukula, JA Beckman, AR Prisco, S Lin, TF Dardas, RK Cheng, JW Smith, NA Mokadam, C Mahr and A Aliseda, Small LV Size is an Independent Risk Factor for VAD Thrombosis, accepted for publication in ASAIO Journal (2018)
-
A Aliseda , VK Chivukula, P McGah, AT Prisco, JA Beckman, GJM Garcia, NA Mokadam and C Mahr, LVAD Outflow graft angle and thrombosis risk, ASAIO Journal 63(1), 14-23 (2017)
-
C Mahr*, VK Chivukula*, P McGah, AR Prisco, JA Beckman, NA Mokadam and A Aliseda, Intermittent aortic valve opening and risk of thrombosis in VAD patients, ASAIO Journal 63(4), 425-432 (2017)
-
Levitt, M.R., Barbour, M.C., du Roscoat, S.R., Geindreau, C., Chivukula, V.K., McGah, P.M., Nerva, J.D., Morton, R.P., Kim, L.J. and Aliseda, A.. Computational fluid dynamics of cerebral aneurysm coiling using high-resolution and high-energy synchrotron X-ray microtomography: comparison with the homogeneous porous medium approach. Journal of neurointerventional surgery, 9(8), pp.00-00.(2016)
-
V. Chivukula, S. Goenezen, M. Midgett, S. Rugonyi, Effect of Outflow Tract Banding on Embryonic Cardiac Hemodynamics. Journal of Cardiovascular Development and Disease 3(1),1, (2016)
-
J. Crawford, V. Chivukula, S. Haller, N Vatankah, C Bohannan, G Moneta, S. Rugonyi, A Azarbal Aortic outflow occlusion predicts rupture of abdominal aortic aneurysm Journal of Vascular Surgery, 64(6) 1623-1628 (2016)
-
M. Midgett, V. Chivukula, C. Dorn, S. Wallace and S. Rugonyi, Blood flow through the embryonic heart outflow tract during cardiac looping in HH13–HH18 chicken embryos. Journal of The Royal Society Interface 12.111: (2015)
-
S. Goenezen, V. Chivukula, L. Phan, S. Rugonyi, 4D subject-specific inverse modeling of the chick embryonic heart outflow tract hemodynamics. Biomech. Model. Mechanobiol: 1-21 (2015)
-
V. Chivukula, B. Krog, J.T. Nauseef, M.D. Henry, S.C. Vigmostad, Alterations in cancer cell mechanical properties after fluid shear stress exposure: a micropipette aspiration study, Cell Health Cytoskeleton. 25–35 (2015)
-
V. Chivukula, J. Mousel, J. Lu, S. Vigmostad, Micro-scale blood particulate dynamics using a non-uniform rational B-spline-based isogeometric analysis., Int. J. Numer. Method. Biomed. Eng. 30, 1437–59 (2014)
-
V.K. Chivukula, Development of a NURBS-based particulate dynamics framework for modeling circulating cells, University of Iowa, Ph.D. Theses Diss. (2014)
-
T.D. Almomani, S.C. Vigmostad, V.K. Chivukula, L. Al-zube, Red blood cell flow in the cardiovascular system: A fluid dynamics perspective, Crit. Rev. Biomed. Eng. 40, 427–440 (2012)
* these authors contributed equally to the manuscript
​
Google Scholar Citation Record
​